EEPS 668 Field Trip Blog
by Gaetano Ferrante, JongGil Park and Kaveh Farnoudi
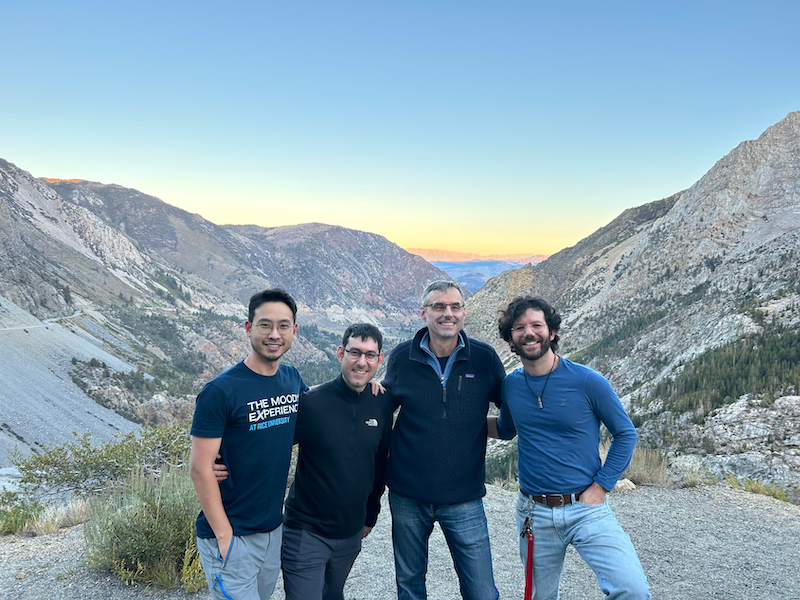
Day 1: Long Valley Caldera and the Bishop Tuff
Stop 1: The Bishop Tuff deposit
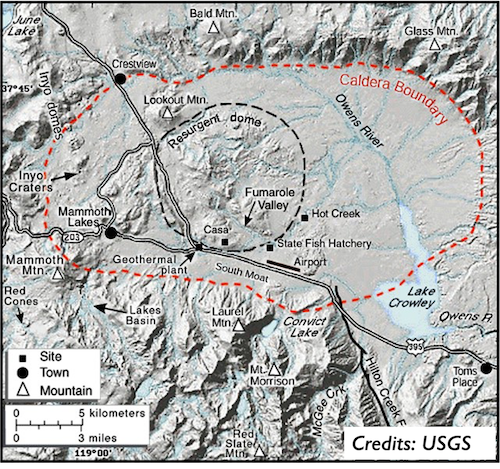
Our trip started in the town of Mammoth Lakes, which served as our headquarters for the first two days of exploration. The first stop was Long Valley Caldera, just outside of Mammoth Lakes (fig. 1). Long Valley Caldera is a 32 km long and 18 km wide depression in eastern California, formed 760,000 years ago during what volcanologists call a “supereruption”. During a supereruption, tremendous amounts of pyroclastic material (pieces of broken magma) are violently ejected into the atmosphere, eventually reaching the ground as pyroclastic falls or pyroclastic flows. During the Long Valley eruption that occurred 760,000 years ago, some 650 cubic kilometers of volcanic ash (fine fragments of broken magma) were released.
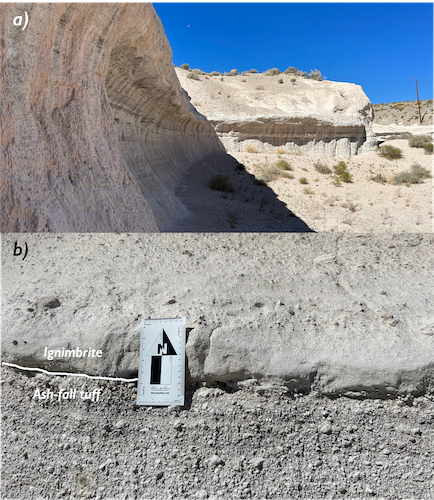
This caused rapid depressurization of the magma chamber, resulting in the catastrophic collapse of the volcano and the formation of a “caldera” (Spanish for cauldron), a large bowl-shaped depression of the ground. The pyroclastic deposits associated with this supereruption are collectively known as the Bishop Tuff (fig. 2a).
Caldera-forming eruptions form sustained columns of pyroclast-laden gases that rise to more than 25 km in the atmosphere. Fall deposits form through gravitational settling of pyroclasts from the eruptive plume, and thus often showcase sorting, grading and bedding. If the eruptive column becomes gravitationally unstable, it can collapse under its own weight, producing avalanche-like flows that travel away from the volcano at hundreds of kilometers per hour. Settling of particles from such pyroclastic flows does not commonly result in sorting or grading, instead producing massive mixtures of pumice fragments, crystals and ash called “ignimbrites”. Both ash-fall deposits and ignimbrites are present in the Bishop Tuff, and the contact between them is usually pretty sharp and easy to identify (fig. 2b).
Stop 2: The Owens River Gorge
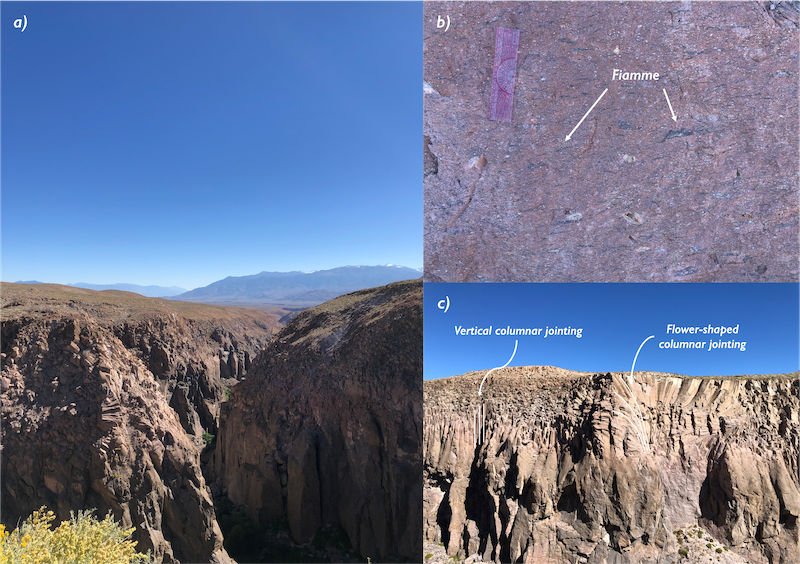
Southeast of the caldera lies the Owens River gorge, the target of our following stop (fig. 3a). This steep-walled valley formed as the Owens River cut through the Bishop Tuff, exposing its internal structure. In this area, the tuff showcases high degrees of welding. If pyroclasts ejected during an eruption are still relatively hot upon falling to the ground, they can weld together, deform and flow. The resulting pyroclastic rock is known as “welded tuff”, and commonly contains an abundant proportion of pumiceous fragments. Near the top of the deposit, where the lithostatic pressure was low at the time of deposition, these fragments preserve their original shape. As we descended deeper into the gorge, the fragments assumed an elongated, flame-like shape (fig. 3b). Such a shape results from compaction of the pore space inside the pumices due to the weight of the overlying rocks, which forced out the gas contained in the vesicles, much like water flowing out of a squeezed sponge. The resulting elongated pumices are known as “fiamme” (Italian for flames), and the resulting rock texture is termed “eutaxitic”.
After emplacing, the hot deposit cooled down. Cooling is associated with contraction, which generates stresses in the confined deposit. These stresses can be high enough to fracture the rock, forming numerous joints. Fracturing, however, implies breaking molecular bonds, for which energy is required. As a consequence, fractures produce hexagonal columns, since (as bees know well) hexagons have the smallest perimeter for the same area among the regular polygons. This phenomenon is called “columnar jointing” (fig. 3c). Fractures grow perpendicular to the planes of constant temperature, so that columnar joints tend to be vertically aligned if the rock only cools by conducting heat to the overlying air and the underlying ground. However, abundant hydrothermal circulation followed the emplacement of the Bishop Tuff, as the hot pyroclastic material vaporized groundwater and released magmatic gases through fumaroles. As they made their way towards the surface, these hot steams distorted the temperature profile, often producing flower-shaped columnar joints. At the surface, these fumaroles are now preserved as mounds of more resistant rocks, likely the result of silica deposition from the condensing steam.
Stop 3: Panum Crater
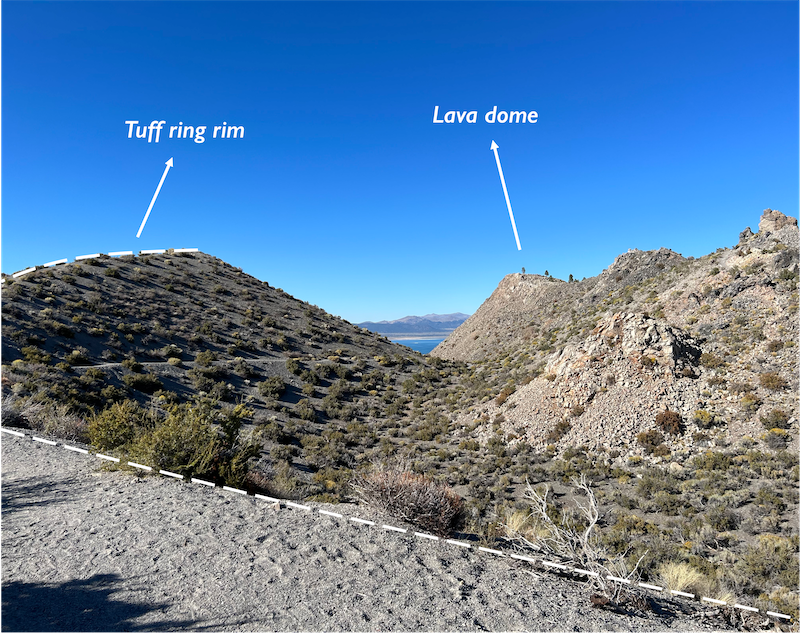
The last stop of the Long Valley section of our field trip was Panum Crater. Panum is the youngest vent of the Mono craters, a chain of some 30 domes, flows and craters associated with the Long Valley volcanic system. The crater has a complex history, punctuated by a succession of phreatic and magmatic eruptions. Phreatic eruptions occur when magma vaporizes groundwater to steam, whose sudden expansion produces explosions that can fragment the surrounding rock, forming a crater and piles of ejected material surrounding it.
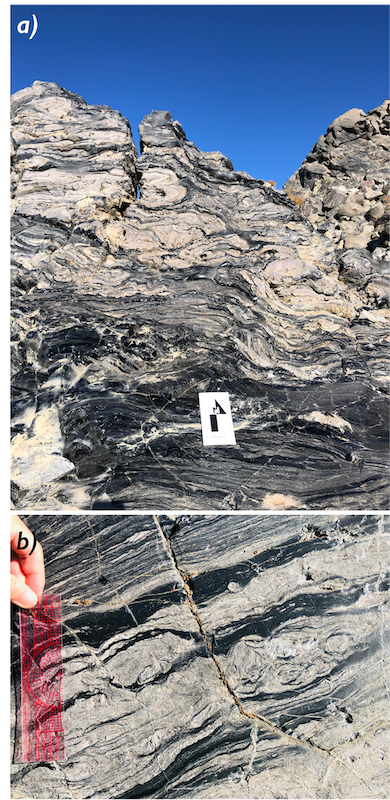
The ejecta ring surrounding Panum Crater was mostly formed by magmatic eruptions following the first phreatic phase (fig. 4). In addition, the center of the crater is now occupied by a lava dome, formed as viscous rhyolitic lava slowly extruded from the vent, like
toothpaste out of its container. The lava dome is made up of pumice and obsidian of the same composition, but showing variable degrees of vesicularity. Stretching of heterogeneities during viscous laminar flow of the magma produced peculiar patterns of alternating pumiceous and obsidian bands, creating a texture that is commonly referred to as “flow banding” (fig 5). It has been speculated that effusion of lava during the extrusion of the dome was accompanied by ejection of gas and pyroclasts from fractures in the lava, which eventually became preserved in the geological record as ash-filled cracks termed “tuffisites”. We turned Panum Crater inside out looking for tuffisites, risked our lives walking on sharp-edged obsidian blocks, but we couldn’t find any.
Day 2: The Tuolumne Batholith
On the second day, after a short stop at Obsidian Dome, which is part of the Mono-Inyo chain, we continued our journey along Hwy 395 to look at granitic outcrops of the central Sierra Nevada, find out about their eruptive history and decipher how this intrusive complex was emplaced during the late Cretaceous.
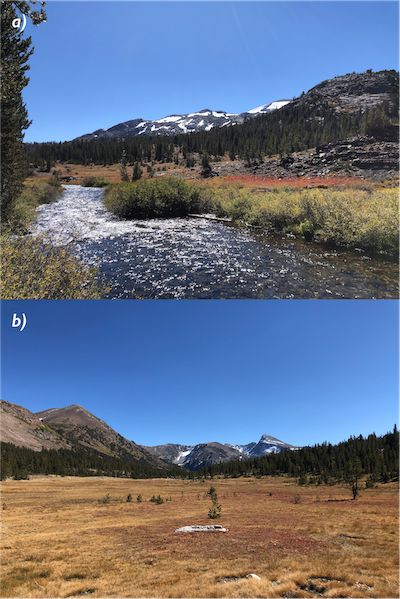
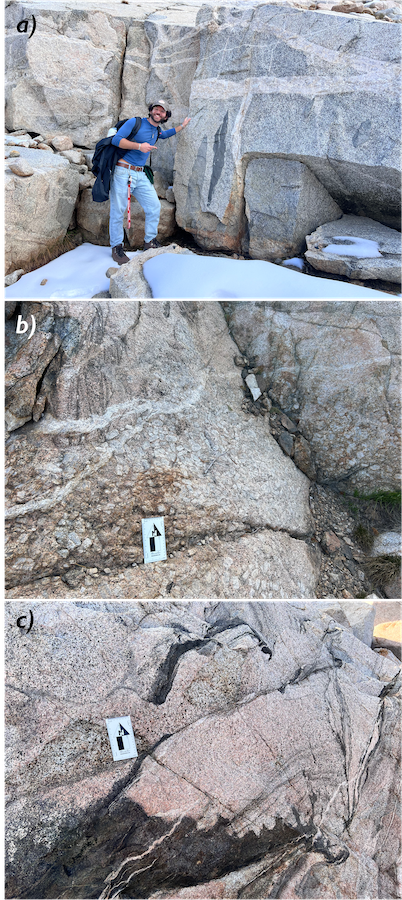
Our final destination was the Tuolumne Batholith, which is exposed west of Mono Lake. A batholith is a large intrusive igneous body formed by the repeated injection and solidification of magma over a large area in the Earth's crust, such as would happen for example along a subduction zone. And it was, in fact, prolonged subduction of the Farallon plate beneath the North American plate during the Cretaceous that provided the magmatic fuel necessary to build up the batholith over time. This turned out to be the longest and most breathtaking hike of our field trip, crowned by the most impressive among the outcrops. It started with us wading across the small Lee Vining Creek (fig. 6a), crossing a meadow (fig. 6b), and soon realizing that we could have taken an easier route. But we eventually made it to the granites, where we indulged in some fun snow sliding (click HERE to watch a video of JongGil sliding).
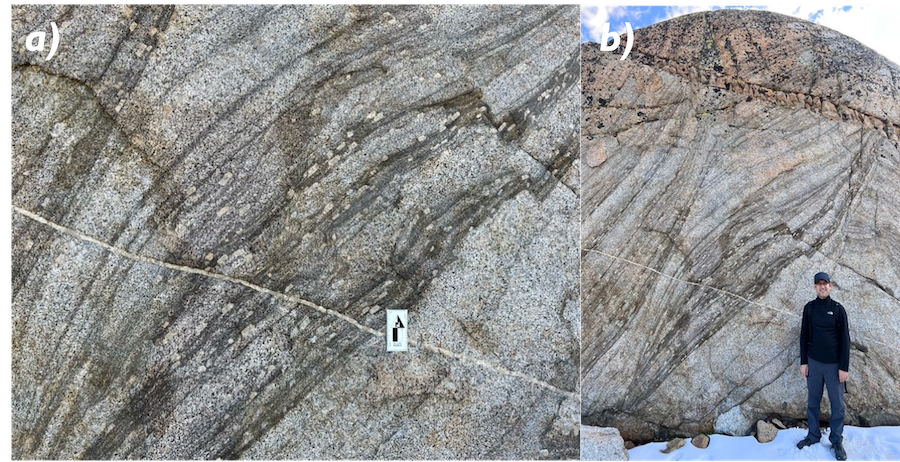
Far from the homogeneous granites looming over Rice's engineering quad, the Tuolumne granites showcase all sorts of heterogeneous structures (fig. 7): mafic and lithic enclaves showing evidence of magma mixing and stoping (erosion of the wall rock by the hot magma); feldspar graveyards reflecting crystallization and settling of huge plagioclase crystals, called megacrysts; dark-colored streaks winding through the light granitic matrix to which volcanologists gave the german name of ”schlieren". It has been proposed that reactions between mafic enclaves and the host magma produce a biotite-rich rind around the enclaves, which can get stretched and deformed during magma mixing, eventually producing the elongated schlieren. Schlieren structures were particularly impressive in the last outcrop of the day, to the point where every single one of us felt the need to appear in one or more pictures standing next to them (fig. 8). On top of that, the outcrop was notable for the presence of feldspar megacrysts aligned with the schlieren streaks, which prompted us to ponder about the processes occurring in the magma chamber during its formation. Thinking about magma mixing made us even more tired, so we decided to head back to our car, this time through the shortest and easiest trail.
Day 3: Red Rock ignimbrites
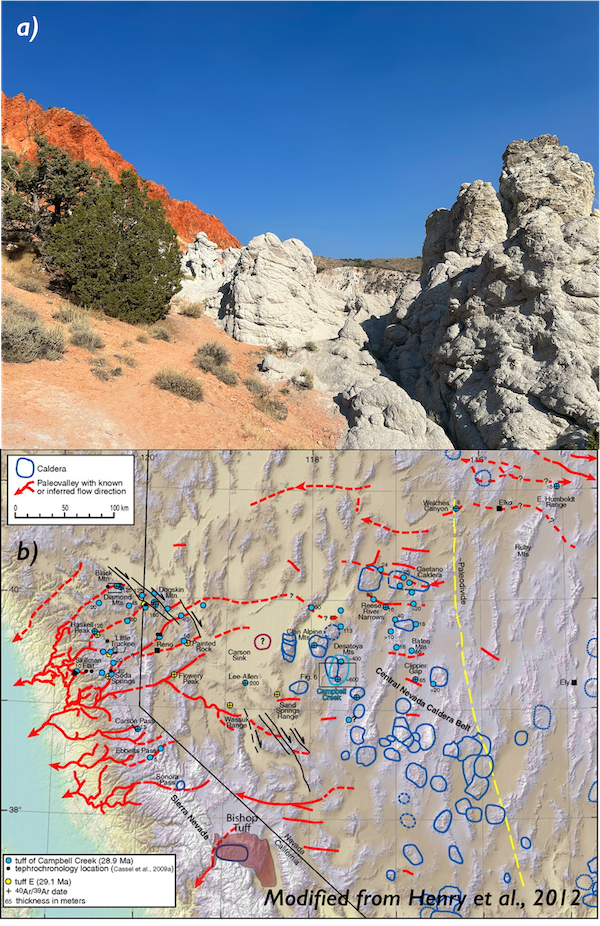
The third day was the day of the long drive north. We first stopped in Carson City, the capital of the State of Nevada, where we celebrated Helge's birthday at a local restaurant. Pizza and BBQ provided us with the right fuel to continue our journey towards Mount Shasta. Before leaving Nevada, however, we met with fellow volcanologist Philipp Ruprecht, who took us on a small detour to some colorful ignimbrites known as Red Rock (fig. 9a). Philipp told us that 40 million years ago Nevada was part of a high-elevation plateau, commonly known as “Nevadaplano”. The Nevadaplano was drained to the Pacific Ocean and across the Sierra Nevada by numerous paleovalleys (fig. 9b).
Between 36 and 18 million years ago, a flareup of explosive silicic volcanism formed about 200 calderas, which flooded southwestern North America with ash flows. These pyroclastic flows were able to travel fast and far, being channelized along the topographic lows of the ancient valleys. Today, ignimbrites such as the Red Rock complex are exposed throughout Nevada, providing geologists with clues on the location of these paleovalleys.
After breaking some rocks with a hammer and admiring more fiamme in the massive ignimbrites, we parted ways with Philipp and resumed our journey towards Mount Shasta.
Days 4 and 5: Medicine Lake Volcano and Glass Mountain
Stop 1: Mount Shasta
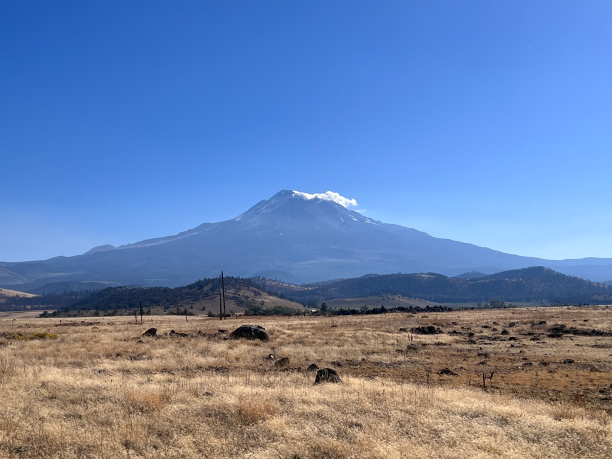
Our last two days were dedicated to Medicine Lake volcano, with its variety of eruptive products. Before reaching the volcano, however, we took a short detour and indulged in a spectacular view of Mount Shasta, the southernmost stratovolcano of the Cascade Range (fig. 10). After all, we were sleeping at its feet in a town that bears its name. After observing the remains of a huge flank collapse that had puzzled geologists for decades, we jumped back on our Suburban and headed towards Medicine Lake.
Stop 2: Lava flows of Medicine Lake Volcano
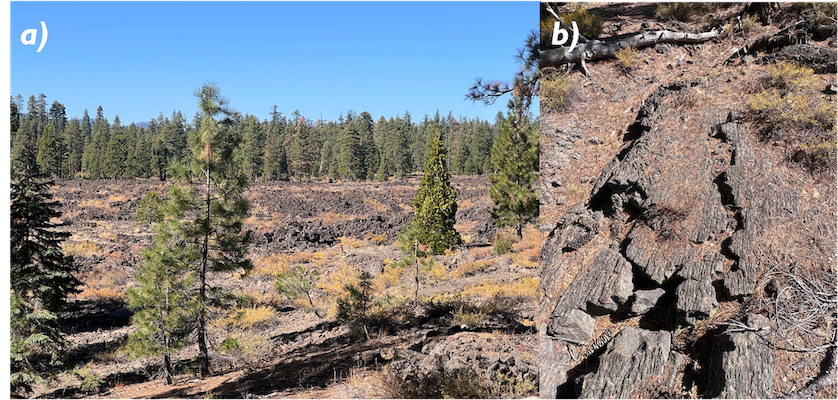
Medicine Lake Volcano is a shield volcano located in northeastern California, 50 kilometers east of Mount Shasta. It is one of the largest volcanoes in the Cascade Range, with a volume of approximately 600 cubic kilometers, and it is of Pleistocene to Holocene age. Unlike volcanoes like Mount St. Helens, which are built through the successive emplacement of viscous lava flows and pyroclastic deposits, Medicine Lake volcano was formed by effusive eruptions, which released the lava flows that now coat its surface. The predominance of low-viscosity, mafic lavas shaped the volcano's gentle slopes, earning him the title of “shield volcano”. Around 100,000 years ago, a series of eruptions resulted in the formation of a caldera on the summit of the volcano. Today, this crater hosts the namesake Medicine Lake. Throughout its history, the volcano has erupted various types of magma, ranging from basaltic to rhyolitic. Medicine Lake volcano also exhibits a wide variety of volcanic features, including glass flows, obsidian deposits, lava tubes, and cinder cones.
While ascending the flanks of Medicine Lake volcano, we encountered two types of basaltic lava flows: pahoehoe lava, which exhibits a smooth, ropy texture, and a‘a lava, characterized by a blocky, spiny surface (fig. 11). Pahoehoe lava forms when magma flows relatively slowly, allowing a skin to develop on the surface, which prevents heat loss. If this
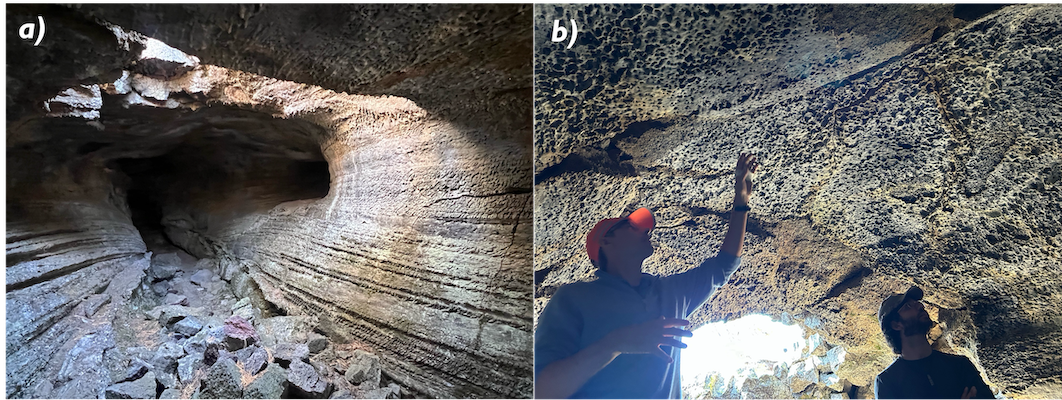
skin is disrupted, the underlying lava flows out to heal the breach, preserving a smooth surface. This surface skin, by thermally insulating the flow, allows the lava to continue flowing in a liquid state, forming lava tubes (fig. 12). When the eruption ceases and the lava drains away, an empty lava tube is left, which often preserves indication of the flow level of the magma as grooves on the lateral walls. On the other hand, in the case of faster flows in open channels, significant heat loss causes increased viscosity, preventing the lava from healing the surface crust and resulting in the rugged and clinkery appearance of a‘a flows. We then headed to Glass Mountain, to observe the two endmembers in the vesicularity of rhyolitic eruptive products: pumice and obsidian.
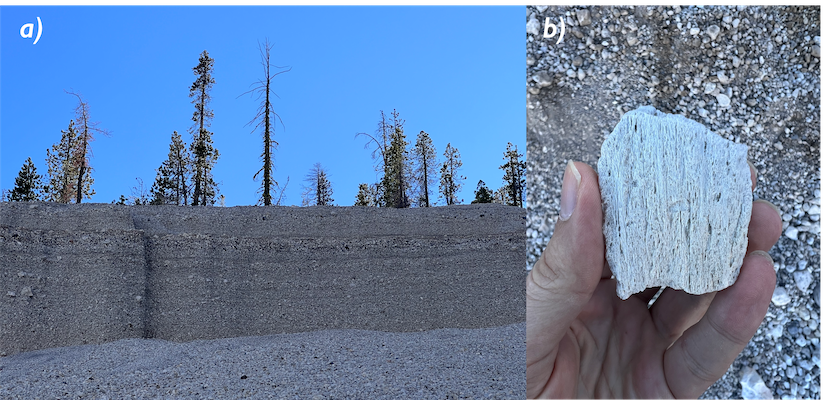
We took advantage of a Sunday morning when no trucks or cranes were operating to visit a pumice quarry. This pumice quarry was predominantly composed of white pumice, interspersed with dark-colored lithic fragments (fig. 13a). The pumice has piled up with an angle of repose of approximately 20-30 degrees. When JongGil attempted to climb the slope, he was swamped by a small pumice landslide, making further progress impossible (click HERE for a video of JongGil sliding on pumice). We therefore surrendered and settled for the pumice fragments at the bottom of the slope. These pumices, created during the 1060 CE explosive eruption of Glass Mountain, are very lightweight and contain a multitude of vesicles. Some fragments had extremely elongated vesicles resembling tubes, earning the name "tube pumices" (fig. 13b). They likely formed due to strong deformation near the walls of volcanic conduits during the eruption, causing bubbles to stretch. As the bubbles elongate, the magma close to the conduit walls experiences localized reduction in viscosity, causing complex feedbacks affecting the velocity profile of the magma in the conduit.
After visiting the pumice quarry, we jumped back in the car and drove around Glass Mountain looking for our next stop. Glass Mountain is a rhyolitic and dacitic obsidian flow that erupted from the eastern caldera rim of Medicine Lake Volcano about 950 years ago. A group of rhyolitic domes lie on a NW-SE trend to the north of the main flow and one to the south, probably the surface manifestation of the dike feeding the eruption. We eventually stopped at the northwestern edge of the flow to observe one of the lava domes in better detail. We parked our car at the side of the road, at the feet of a steep tephra cone made up of coarse pumice fragments. Due to the steepness of the slope and the sharpness of the fragments, we had to wear gloves and proceeded to cautiously climb the cone. When we eventually made it to the top, we discovered a lava dome towering from its center (fig. 14). We decided to walk around the rim of the cone, trying to investigate the relationship between the obsidian dome and the tephra deposit. Every now and then, we stumbled upon meter-sized, partially fragmented blocks of obsidian lying on the pumiceous tephra cone. We interpreted them as volcanic bombs, large fragments of magma ejected following ballistic trajectories during volcanic eruptions. We hypothesized that the tephra ring surrounding the lava dome resulted from Vulcanian eruptions that disrupted pre-existing lava domes, similar to the one that we observe now and which was not fragmented, unlike its predecessors.
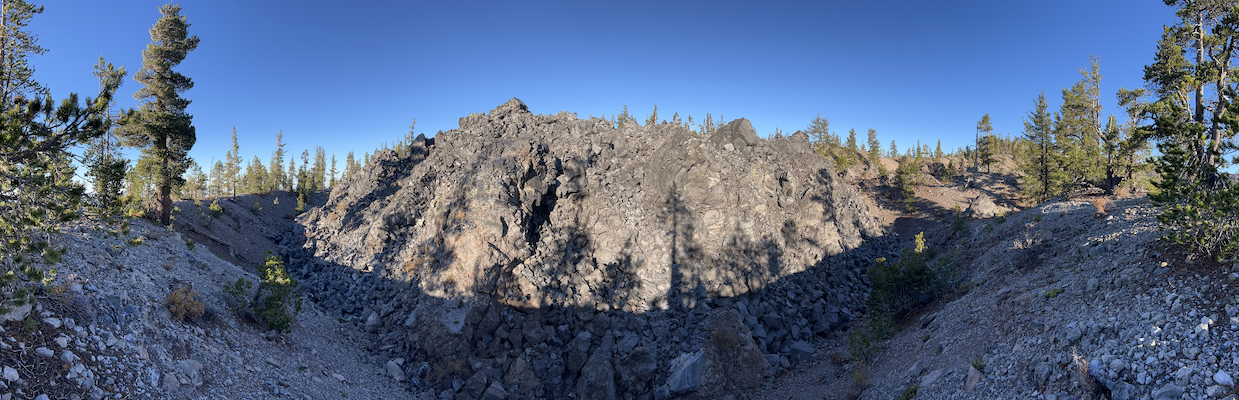
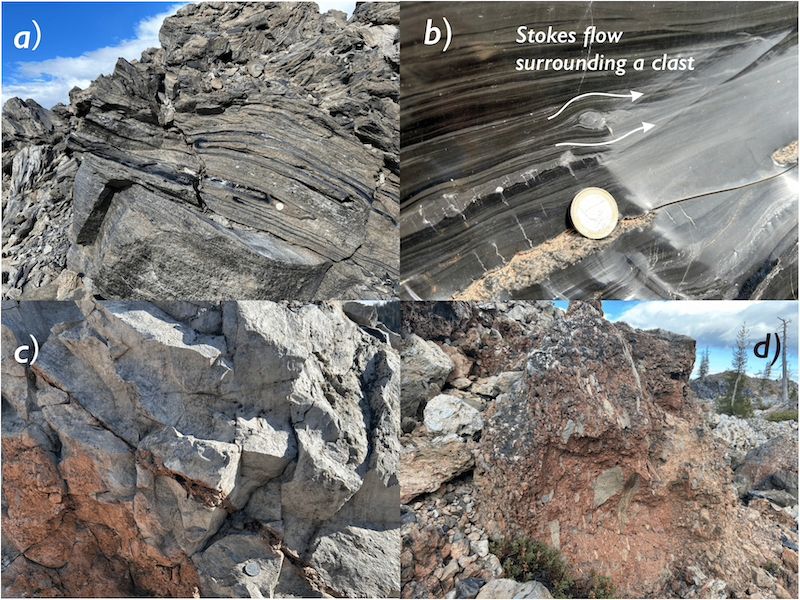
The next day, we revisited Big Glass Mountain and parked at its south lobe. Obsidians nearby the road were piled up steeply. As we ascended along the glass slope, at each step, the obsidian rolled down, creating a sound that resembled the sound of sweeping broken glass with a broom (listen to audio of glass moving). Fortunately, there was a track that made it easy to hike all the way up to the top of the flow, where we could see the entire region, showing uneven surfaces with small puddles of obsidian. We stayed there for about 3 hours to find interesting features of obsidian, like treasure hunters. Many obsidian pieces exhibited flow banding, with lighter bands reflecting higher vesicularity (fig. 15a). Additionally, there were traces of small enclaves within the obsidian and evidence of flow patterns (fig. 15b). In one of the small pits, a feature resembling a tuffisite, which we had not previously discovered in the Long Valley Caldera, was found (fig. 15c). The obsidian showed evidence of fractures, with the interior filled with pyroclasts of various sizes that have stuck together, and the interior exhibited a reddish color due to oxidation. However, unlike the tuffisite formed by pyroclasts within fractures of the conduit sticking together due to their momentum, in a location slightly farther from the vent, agglutinated features could be observed (fig. 15d). These represent pyroclastic deposits that have been ejected from the conduit, still hot and viscous, fusing together outside the conduit.
Stop 6: Little Mount Hoffman
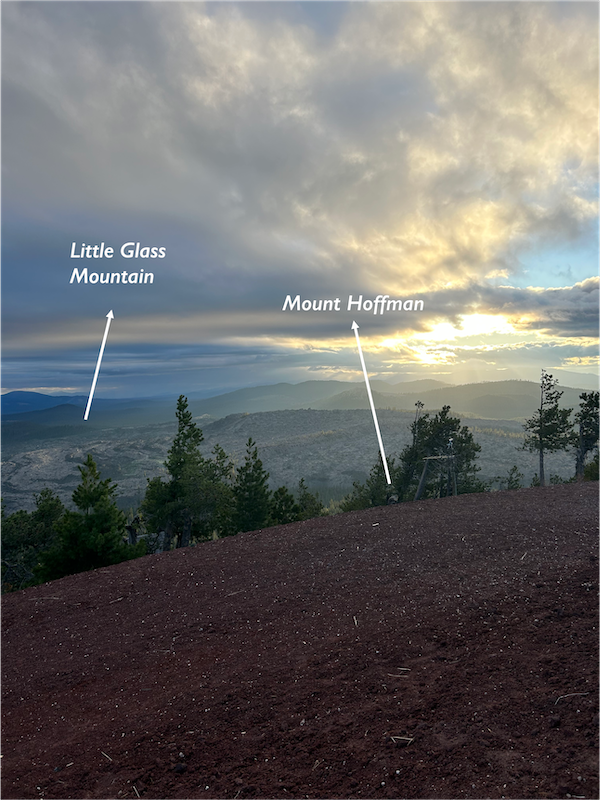
Before heading back home, we concluded our last day with a stop at Little Mount Hoffman. Little Mount Hoffman is a cinder cone located about 1 kilometer away from Little Glass Mountain, and its slope is approximately 30-40 degrees. At the base of Little Mount Hoffman, we observed light-colored pumice and reddish scoria mixed on the slope of the cinder cone. However, as we ascended to the summit, the proportion of scoria increased, and, at the top, the cone was composed entirely of red scoria. (fig. 16). The vast textural and compositional differences between the two rock types initially left us puzzled. After some thinking and brainstorming during our drive back home, we figured out that the pumice and the scoria belong to separate eruptions. First, the red scoria was deposited during a monogenetic episode of basaltic fire-fountaining, which formed the Mount Hoffman cinder cone. Subsequently, a subplinian eruption of Little Glass Mountain blanketed the whole area with the light-colored pumice, with some also being deposited on Mount Hoffman. However, the pumice deposited on the summit of the cinder cone may have rolled down to lower areas due to gravity, leaving mainly scoria behind.